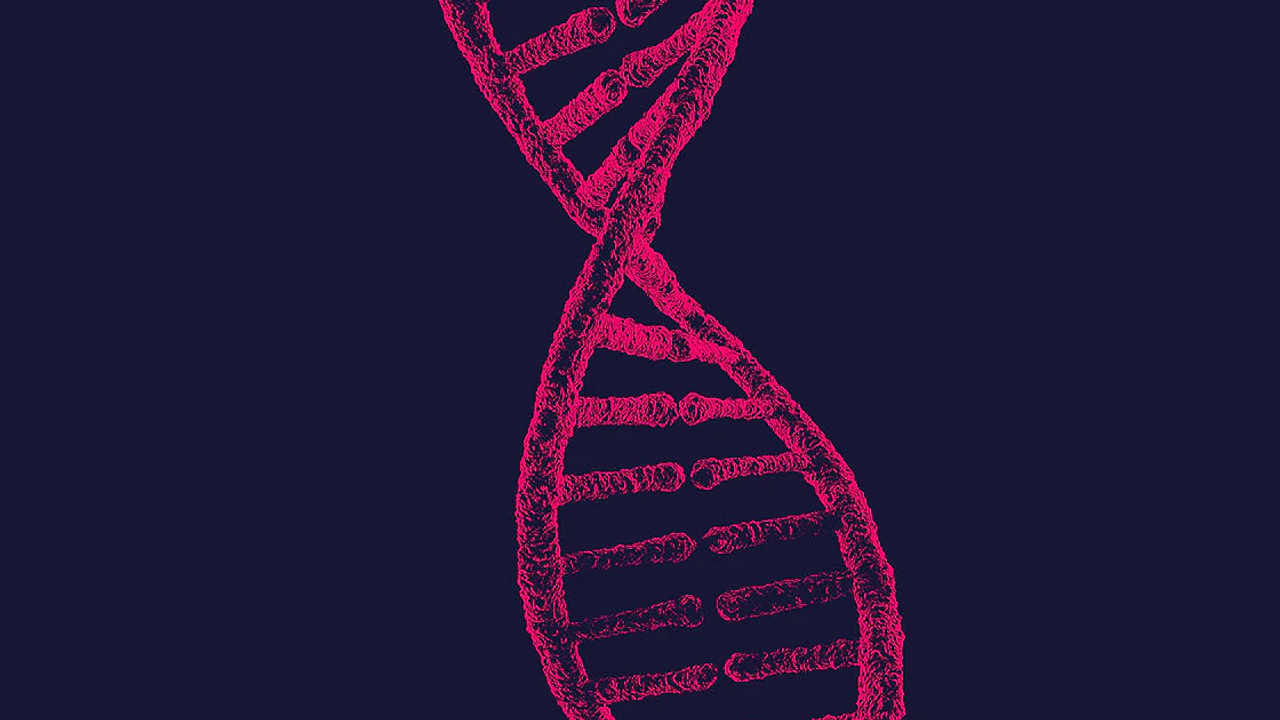
Gene Expression
Jun 24, 2022Video on sitting biomechanics: Click Here
The selfish-gene model is the one we learned in high school. It explains that for almost every population, genes create traits and drive evolution. This idea comes from Gregor Mendel and all of his work on peas done in the 1860s (Miko, 2008). Although Mendel didn’t expose the physical gene itself, he still conceptualized it. His data showed that traits appeared, or disappeared, in consistent ratios dictated by mathematical formulas (Mclean, 2000). This math simply explained how inheritance works. All of this was packaged and first published in 1866, which was only 7 years after Charles Darwin published On the Origin of Species. Because of this, no one paid much attention to Mendel’s work. It wasn’t until the 1900s that biologists began to dig it up. The term ‘gene’ was then officially established in 1909, which filled a large hole in Darwin’s theory of evolution (Portin & Wilkins, 2017). This was one of the most critical discoveries in biology which led scientists to believe that genes can ultimately explain everything.
Darwin only proposed the mechanism in which evolution occurs as technology during his time limited him from understanding the fundamentals of molecular biology. The discovery of the gene explained the basis of heredity. Although, one of the main concerns for scientists now was how it didn’t explain altruism. Why do humans, and other animals, help one another? This question was approached mathematically. They observed and calculated patterns in relation to how animals act generously in ways to aid others such as their offspring, parents, siblings, cousins, tribal mates, etc (Okasha, 2003). Animals express altruism in ways to help share their genes—the closer they are, the kinder and more altruistic they behave (Okasha, 2003). This is what reconciled Mendel and Darwin’s work to establish modern genetics and evolutionary theory as one. The discovery of the structure of DNA topped it all off in which it was able to explain the transformative properties of the gene.
To take a step back and lay out these properties, we need to start with the chromosome. The chromosome is the physical structure in all cells that contains the genes. It conveys genes between both the generations of dividing cells, as well as the generations of whole organisms (Finegold, 2021). These genes are what provide the cells with the essential information needed to build and maintain themselves. Each chromosome at its core has a single, unbroken molecule of DNA. These structures can contain hundreds to thousands of genes arranged in a chain (Annunziato, 2008). Humans have 46 chromosomes, and in chromosome number 2, for example, there are over 1,300 different genes alone. If we were able to combine and then stretch out all of the DNA inside our genes, it is said to be long enough to stretch from Earth to the sun and back 65 times (20 billion km long) (Nurse, 2021, 22).
Our genes are made of DNA. Each and every gene in your body is a defined stretch of the DNA code that contains an instructional message for the cell (Donohue, 2022). The structuring of this DNA code comes in the form of a double helix structure. This structure is what was proposed by Watson and Crick in 1953. This structure is what will help us understand more about the fundamentals of the gene as a unit. First, the DNA structure encodes information that cells and whole organisms need to grow, maintain, and reproduce themselves (Cooper, 2000). Second, DNA must be able to replicate itself, perfectly and precisely, allowing each new cell and each new organism to inherit a complete set of genetic information. We can think of the DNA helical structure as a twisted ladder designed to carry information. The side rail is the sugar-phosphate backbone of the helical structure, while the rung of the ladder is made from links that form the pair of chemical molecules known as nucleotide bases. The bases come in a set of four: Adenine, Thymine, Guanine, and Cytosine. These four molecules arrange themselves in a similar way we would understand the coding of a computer. Adenine and Thymine are base pairs (A, T), while Guanine and Cytosine are base pairs (G, C). A can only pair with T, and G can only pair with C. This is the foundation of the informational coding embedded in our genes. These arranged letters are the encoded instructions that determine everything from eye color to height (Nurse, 2021).
The cell takes these instructions from the DNA by ‘reading’ this genetic code. How the cell ‘reads’ this information determines how the cell uses this information. This allows us to understand that the gene is what instructs the cell on how to construct particular proteins—which is vital to life as protein makes up our cell's enzymes, structure, and operating systems. Therefore, we have two programs for the cell to operate: the first is the four-letter alphabet of DNA made up of the letters A, T, G, C, while the second is the ordered strings of the 20 different building blocks known as amino acids. This relationship between genes and proteins is known as the ‘genetic code’. This is all foundational for the selfish-gene model which states how the gene drives behavior. Which we now know is simply not true. According to Michael Eisen, an evolutionary biologist, the gene’s power to create traits is just one of many evolutionary mechanisms. It’s not so much that the genes don't drive evolutionary change, it's just that changing the gene, to change the organism, is only one way to get the adaptive job done, but in reality, there may actually be better ways to do this.
Human cells are eukaryotic, which simply means that they contain organelles, like a nucleus (Manning, 2017). Everything we just discussed about the gene is happening within the nucleus. We have around 25,000 genes within our 46 chromosomes. All 25,000 genes are present in every single one of our cells, but the thing is, not all of our cells are the same. We have muscle cells, bone cells, blood cells, nerve cells, skin cells, etc (Baxter, 2022). The difference between these cells is that different genes are being expressed at different times (O'Connor, 2011). Each cell has to determine which genes it wants to put to use. This is what we consider gene expression. The gene is simply the instructions, which is what we call the genotype (Taylor & Lewontin, 2017). This set of instructions then gets made into protein, which is the phenotype. The genotype is the instructions to the phenotype—which is the physical appearance.
An example of this is shown in grasshoppers—which also have eukaryotic cells. Grasshoppers display gene expression that leads to complete transformation. If you have never seen a locust, it would not make this transformation as impressive. The reason being is that the locust and the grasshopper look quite different—like comparing a grasshopper and cricket. You might not even think these insects are related at first glance, but not only are they related, they're also genetically identical (Dobbs & Hains, 2013). The only difference between the two is how the genes are being read. Basically, how it happens is through a spike in serotonin levels which changes the gene expression so much that it alters not only the grasshopper's behavior but also its appearance and form. The brain quite literally grows to manage the socially acquainted world of a locust. This transformation can happen within hours. And the cause of it is the environment. If the grasshopper is placed in an environment with a low food supply, it will transform into a scourged locust. It can return back to the grasshopper just as quickly as it turned into the locust, too. This is just one example as there are many more. But the question is, how does this happen?
The following is the cell membrane theory leading to gene expression, known as “the central dogma of molecular biology”. There are currently alternate theories accounting for the separation from the extracellular to intracellular environment, receptors, etc. These will be described in the follow-on literature. Gene expression is complex. To understand this, we must once again look closely at the cell. In the external environment of a cell, proteins interact with specific receptors on the cell's outer membrane (Alberts, 200). This triggers a cascade of events leading to the expression of genes which then leads to protein production. The once thought “one gene to one protein” theory has been found to be incorrect. Cells use alternative splicing to produce over 100,000 proteins from 20,000 genes.
The outer membrane is regulating what comes in and what stays out. The receptors on the surface of the membrane trigger signal transduction involving other proteins in the cell's cytoplasm. Within the cell's cytoplasm, the signaling elements trigger another cascade of events in the cell’s nucleus. This final component interacts with the DNA in the nucleus at very specific sites to begin the expression of the desired gene which begins a two-part process. The first is called transcription, which is when DNA is copied into a messenger RNA (mRNA). This mRNA then has to undergo many changes, which include the removal of RNA receptors not needed to code for the desired proteins. The mRNA returns to the cytoplasm where the second part of protein production, translation, occurs.
Ribosome RNA (rNA), then forces the interaction with the mRNA and transfer RNAs (tRNAs), which brings the amino acids from the cytoplasm to the ribosome, to create the polypeptide chain of amino acids. This protein then undergoes changes in a configuration including specific folding. Finally, each protein takes on a 3D shape as a functional end product which then leaves the cell to take on its role in the body (Alberts, 2001).
As I said, gene expression is complex. It all starts on the outside of the cell via protein interaction with the cell's outer membrane. The proteins are essentially recruiting specific genes for the production of a certain protein. In mammalian genomes, DNA methylation is the epigenetic mechanism known to regulate gene expression by either recruiting proteins involved in gene repression, or by inhibiting the transcription process (Li, 2014). Some of the highest levels of DNA methylation are located in the brain (Moore, 2013). Neurons (brain cells) react to the environment through patterns of depolarizations which relay information to the brain and encode a response. Research in recent years is suggesting that following depolarization, alterations in gene expression are accompanied by modifications of the epigenetic cascade including alterations in the pattern of DNA methylation. DNA methylation is essential for regulating tissue-specific gene expression (Wan, 2015). Recent studies on human tissues have shown that methylation of the region near the transcription start site is highly informative of gene expression. In the cells of plants and animals, most of the gene sequences are broken up by one or more introns (Brown & Brown, 2002). Many researchers are now suggesting the first intron to be the informative gene feature regarding the relationship between DNA methylation and gene expression (Anastasiadi, 2018).
Recent studies have also revealed, in the European sea bass genome, a clear inverse relationship with gene expression within the first intron. This was shown across all vertebrate species, including two healthy human tissues. The researchers of this study concluded that “there seems to be a conserved role for the DNA methylation of the first intron across tissues, vertebras, and developmental stages.” Much is already known about how the transcription machinery works around the site of initiation in which it starts transcribing. This is officially where the selfish-gene model falls apart as gene expressive observations are very difficult to reconcile with this selfish-gene model. These introns expose a large gap in our understanding of gene expression, but could also be a tool for maximizing protein production.
Most of the transcribed genes in eukaryotic cells get interrupted by introns through the process known as splicing (Clancy, 2008). This alternative splicing is said to increase protein diversity as one of the intrinsic mechanisms for fitness in the varying environment or internal development (Zalabak, 2020). Instead of duplicating the genetic materials, higher organisms can take advantage of alternative splicing as a means to increase protein diversification. This could explain how methylation and other alterations to the chemical wrappings around the DNA establish epigenetic changes. The DNA expression is modulated without the sequence being changed (Kliman, 2016). All of which we can apply to muscle adaptation as most of us already know that our muscles are made of proteins.
The muscle fiber cell is composed of myosin and actin, which are, essentially, force generators in the cell (Cooper, 2000). They require the use of other proteins to generate energy and maintain cellular integrity. Thus, muscle fibers are composed of multiple types of protein molecules. Therefore, we can consider protein slicing as muscle adaptation, but in relation to the expression of genes that are called upon by the environmental stimuli. This allows us to postulate that the genes expressed in my hamstring tissues were being expressed differently in expression A verse expression B. Genes can be mechanosensitive which determines how they are activated (Nowlan, 2008).
Now, before we continue, allow me to clarify that we are only speaking about contact. We are specifically referring to mechanotransduction–we are not discussing other sensory processes that would cause signal cascades at the cell level. This is a theory on mechanotransduction and mechanical stimuli causing DNA transcription and translation for specific protein production.
The mechanics and architecture of the extracellular matrix (ECM) directly impact our tissues (Yue, 2015). Cells in contact with a stiff surface (such as a chair) will typically develop discrete multi-protein complexes under the membrane. These are called focal adhesions—large macromolecular assemblies through which mechanical force and regulatory signals are transmitted between the extracellular matrix and an interacting cell (Kelly, 2020). This occurs when the cellular membrane and ECM make direct contact. These mechanical cues then get transferred to the cellular cytoskeleton. The cytoskeleton is a dynamical structure of interlinking protein filaments in the cytoplasm of all cells. It provides mechanical support to the cells and controls motility, shape, and tension homeostasis (Fletcher, 2010). These mechanical properties of the cytoskeleton depend on the geometry and polarity of its components. The disruption of the cytoskeleton organization is what is said can lead to changes in gene expression—as well as the consequential alterations of cellular response (Tamada, 2004). This all gives us the process from the chair to the nucleus.
The chair creates the mechanical cue, via a compression force, which is perceived by the cells leading to a transfer of information at the site of the extracellular matrix (ECM), via focal adhesions. Focal adhesions receive this information by perceiving the modifications that arose within the extracellular matrix and propagating it at the cytoskeleton level via the interaction of integrin and adapter proteins found on the cell cytoskeleton. This transmission impacts proteins residing at the membrane or in the cytoplasm which can induce their structural modification and subsequent shuttling to the nucleus, as well as signaling from stretch-activated channels that allow ion flow by triggering action potentials, known as the membrane tension model. The ion and protein cascades signal the nucleus leading to protein production. Therefore, we have shown how mechanotransduction leads to protein production.
This concept brings an entirely new perspective to how our body is adapting to the environment. It also brings a different perspective to muscular imbalances. The cells in those tissues are being used completely differently than their intended design. And as we said earlier, some of the highest concentration of methylation is within the tissues of the brain. Therefore, the brain is also a part of how the genes are being expressed–not just the body. Just because I wasn’t sitting on my sit bones in expression A doesn’t mean that my brain actually knew that I wasn’t on my SIT bones. This is the essence of our proprioceptive system. My brain very well could have thought my SIT bones were more near my tailbone than their actual placement. Therefore, the way my genes were expressed was not just solely related to how I was slouching in the chair. The fact that my brain thought this was the correct posture could help explain why my musculature system was unbalanced.
This concept is not new and has been captured in neurological research in which they consider the phenomena a ‘pain map’. The research revealed the organization of sensory maps in the brain that were altered (Cepelewicz, 2021). This is actually quite easy to conceptualize. Consider that you are holding one of your hands out in front of your face. Your focus, attention, and visual system is all attuned to your hand right now. Now, what if all of these sensory systems capturing your hand in space (proprioception) were off? What if your hand was actually located 2 inches to the left but the imagery that your brain is creating has your reality shifted to make it look like you're aligned when in reality you’re not. Therefore you carry out your day thinking that you have full control and understanding of where your hand is when you really don’t—it is actually located 2 inches to the left of where you think it is. How many mistakes do you believe you will make throughout the day? Every time you reach for something, you will miss it. And you won’t know why. Now apply this conceptualization to the SIT bones. My brain, in expression A, could have been fully convinced that my SIT bones were located in that area near my tailbone. Was my proprioceptive system so off that my brain was missing my SIT bone by inches? Not centimeters, but inches? The cells are responding to the subjectivity within the brain. Therefore, if something is real in the brain, it’s real to the body. The movie playing inside our minds that no one besides ourselves can see is objectively real to our body–so it seems.
This is why our behavior in the environment is so important for us to understand. We as humans can consciously control our behavior, which is not to be said about the rest of the animals in the animal kingdom. Their behavior is driven by evolution–it is objective.
Whereas our behavior is subjective.
Written by: Jordan Ettner
References
Alberts, B. (2001). Molecular Biology of the Cell, 4th edition.
Anastasiadi, D. (2018, June 29). Consistent inverse correlation between DNA methylation of the first intron and gene expression across tissues and species - Epigenetics & Chromatin. Epigenetics & Chromatin. Retrieved June 24, 2022, from https://epigeneticsandchromatin.biomedcentral.com/articles/10.1186/s13072-018-0205-1
Annunziato, A. (2008). DNA Packaging: Nucleosomes and Chromatin. Nature Education. https://www.nature.com/scitable/topicpage/dna-packaging-nucleosomes-and-chromatin-310/
Baxter, R. (2022). Types of cells in the human body. Kenhub. Retrieved June 24, 2022, from https://www.kenhub.com/en/library/anatomy/types-of-cells-in-the-human-body
Brown, T. A., & Brown, T. A. (2002). Genomes. BIOS Scientific.
Cepelewicz, J. (2021, August 24). Mental Phenomena Don't Map Into the Brain as Expected. Quanta Magazine. Retrieved June 24, 2022, from https://www.quantamagazine.org/mental-phenomena-dont-map-into-the-brain-as-expected-20210824/
Clancy, S. (2008). RNA splicing: introns, exons and spliceosome. Nature Education. https://www.nature.com/scitable/topicpage/rna-splicing-introns-exons-and-spliceosome-12375/
Cooper, G. M. (2000). The Cell: A Molecular Approach. ASM Press.
Dobbs, D., & Hains, B. (2013, December 3). The selfish gene is a great meme. Too bad it's so wrong. Aeon. Retrieved June 24, 2022, from https://aeon.co/essays/the-selfish-gene-is-a-great-meme-too-bad-it-s-so-wrong
Donohue, C. (2022, June 22). Genetic Code. National Human Genome Research Institute. Retrieved June 24, 2022, from https://www.genome.gov/genetics-glossary/Genetic-Code
Finegold, D. N. (2021, August). Genes and Chromosomes - Fundamentals. Merck Manuals. Retrieved June 24, 2022, from https://www.merckmanuals.com/home/fundamentals/genetics/genes-and-chromosomes
Fletcher, D. A. (2010). Cell mechanics and the cytoskeleton - PMC. NCBI. Retrieved June 24, 2022, from https://www.ncbi.nlm.nih.gov/pmc/articles/PMC2851742/
Kelly, C. (2020, July 13). Phosphoinositides regulate force-independent interactions between talin, vinculin, and actin. PubMed. Retrieved June 24, 2022, from https://pubmed.ncbi.nlm.nih.gov/32657269/
Kliman, R. M. (Ed.). (2016). Encyclopedia of Evolutionary Biology. Elsevier Science.
Li, E. (2014, May). DNA Methylation in Mammals - PMC. NCBI. Retrieved June 24, 2022, from https://www.ncbi.nlm.nih.gov/pmc/articles/PMC3996472/
Manning, A. (2017, November 8). Not so different after all: Human cells, hardy microbes share common ancestor: A research team has found striking parallels between how archaeal and eukaryotic cells package and store their genetic material. ScienceDaily. Retrieved June 24, 2022, from https://www.sciencedaily.com/releases/2017/11/171108092357.htm
Mclean, P. (2000). Mendelian Genetics. Mendelian Genetics. Retrieved June 24, 2022, from https://www.ndsu.edu/pubweb/~mcclean/plsc431/mendel/mendel1.htm
Miko, I. (2008, 1 1). Gregor Mendel and the principles of inheritance. Nature Education. https://www.nature.com/scitable/topicpage/gregor-mendel-and-the-principles-of-inheritance-593/
Moore, L. (2013). DNA Methylation and Its Basic Function. Neuropsychopharmacol. https://www.nature.com/articles/npp2012112#citeas
Nowlan, N. (2008, December 26). Identification of Mechanosensitive Genes during Embryonic Bone Formation. PLOS. Retrieved June 24, 2022, from https://journals.plos.org/ploscompbiol/article?id=10.1371/journal.pcbi.1000250
Nurse, P. (2021). What is Life? Five Great Ideas in Biology. W.W. Norton.
O'Connor, C. (2011, September 8). Essentials of Cell Biology. Retrieved June 24, 2022, from https://web.iitd.ac.in/~amittal/SBL101_Essentials_Cell_Biology.pdf
Okasha, S. (2003, June 3). Biological Altruism (Stanford Encyclopedia of Philosophy). Stanford Encyclopedia of Philosophy. Retrieved June 24, 2022, from https://plato.stanford.edu/entries/altruism-biological/
Phillip, T. (2008). Genetic signaling: Transcription factor cascades and segmentation. Nature Education. https://www.nature.com/scitable/topicpage/genetic-signaling-transcription-factor-cascades-and-segmentation-1058/
Portin, P., & Wilkins, A. (2017, March 24). The Evolving Definition of the Term “Gene”. NCBI. Retrieved June 24, 2022, from https://www.ncbi.nlm.nih.gov/pmc/articles/PMC5378099/
Tamada, M. (2004). Activation of a Signaling Cascade by Cytoskeleton Stretch. Science Direct. Retrieved June 24, 2022, from https://doi.org/10.1016/j.devcel.2004.08.021
Taylor, P., & Lewontin, R. (2017, June 6). The Genotype/Phenotype Distinction (Stanford Encyclopedia of Philosophy). Stanford Encyclopedia of Philosophy. Retrieved June 24, 2022, from https://plato.stanford.edu/entries/genotype-phenotype/
Wan, J. (2015, February 5). Characterization of tissue-specific differential DNA methylation suggests distinct modes of positive and negative gene expression regulation - BMC Genomics. BMC Genomics. Retrieved June 24, 2022, from https://bmcgenomics.biomedcentral.com/articles/10.1186/s12864-015-1271-4
Yue, B. (2015). Biology of the Extracellular Matrix: An Overview. NCBI. Retrieved June 24, 2022, from https://www.ncbi.nlm.nih.gov/pmc/articles/PMC4185430/
Zalabák, D. (2020, July 19). First Come, First Served: Sui Generis Features of the First Intron. PubMed. Retrieved June 24, 2022, from https://pubmed.ncbi.nlm.nih.gov/32707681/